The Future of Chemicals is Electric
- Catalyst
- Oct 11, 2024
- 12 min read
Updated: Oct 23, 2024
Electrochemistry is the key to fossil-free chemical production and offers sustainability benefits that traditional thermochemical systems can’t deliver.
Thousands of everyday products are made from carbon extracted from fossil fuels
Carbon is the building block of our modern material world, the feedstock for chemical production. If the materials we make from fossil fuels were to vanish today, we would be left with a world looking very similar to the medieval world. We would not have the fuels to power transportation or the materials that make up thousands of products we take for granted in daily life, from our favorite running shoes to personal electronics and health-saving devices2. In fact, 96% of all manufactured goods rely on chemical processes or products.
Carbon is the building block of our modern material world, the feedstock for chemical production...In fact 96% of all manufactured goods rely on chemical processes or products.
Carbon is a wonder element, the problem is that for over a century we have been mining carbon in the form of fossil fuels, depleting a limited resource that will inevitably run out and creating greenhouse gas emissions that, by some estimates, account for up to 18% of global emissions. So how do we transition away from fossil fuels and still produce the essential chemicals, materials, and fuels we need? Electrochemistry.
Thermochemistry is the basis of the vast majority of chemical processes we depend on today
The carbon in fossil fuels is in a high-energy state that makes it especially valuable since this form of carbon is easy to refine into fuels for power or into countless chemicals3. It is fundamentally easier to lower the energy of molecules (commonly called chemical energy) than it is to add it back in, as lowering the energy can be as simple as letting the chemical get warm enough to burn in air. A flame is a chemical reaction where the energy of the molecules reacting with each other becomes lower and the lost chemical energy comes out in the form of heat and light.
When materials containing carbon are burnt, the lowest-energy molecule that is produced is usually carbon dioxide (CO2), which is why this is such a pervasive emission from a wide variety of chemical processes. It is simply the most stable form of carbon4, so most of the carbon around us eventually ends up in that form. Going in the opposite direction is more complicated as, now, energy has to be directed back into the molecule to bring it back to the higher-energy, more unstable state. One example of this is CO2 reduction, where CO2 is heated up to temperatures high enough to melt glass5,6 to convert it into the slightly higher-energy carbon monoxide (CO) molecule. In this process, energy is added back into CO2, employing heat as the energy source, and can be thought of as combustion in reverse.
Both a flame and CO2 reduction are examples of thermochemical reactions, which are defined as reactions where the energy difference is transmitted in the form of heat. Thermochemistry is the basis of the vast majority of chemical processes we depend on today. It is possible to produce jet fuel, gasoline, plastics, etc. without fossil fuels by using heat to add chemical energy into CO2, but this requires heat. And the funny thing is, most of the time, we use fossil fuels to produce the heat we need, which takes us back to square one. It is possible to burn materials other than fossil fuels to create the heat energy needed7 but then we need a source of high-energy chemicals that will not run out like fossil fuels, i.e., a renewable resource.
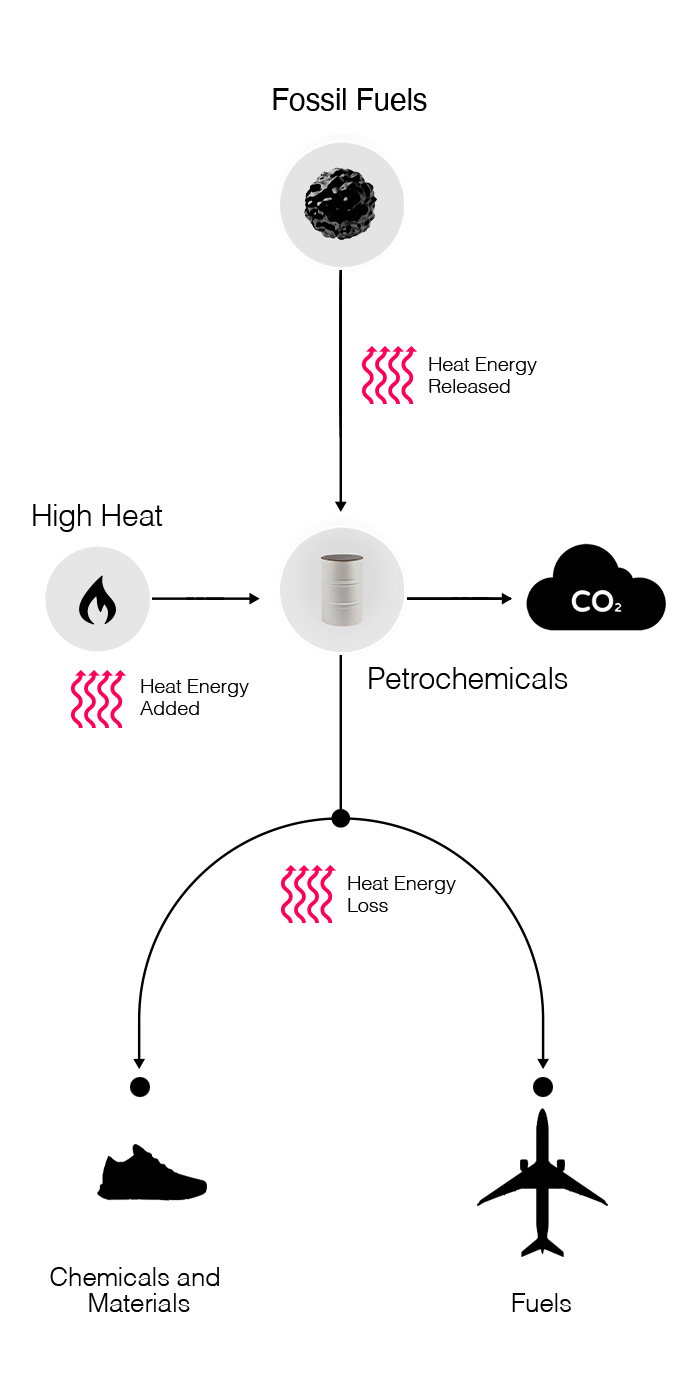
Biologically-derived materials such as plants, wood, and sugar are high in chemical energy and can be used as either fuel or be converted into useful chemicals, just like what is done with fossil fuels. Unlike fossil fuels, biomaterials are a renewable resource. Biomaterials can be viewed as carbon recycling, where photosynthesis converts the low-energy carbon in CO2 into the high-energy carbon that makes up biomaterials. Unfortunately, we may never be able to produce enough biomaterials to cover all the world’s needs. All the energy from sunlight that gets captured by photosynthesis to produce biomaterials adds up to 140 terawatts8, which represents most of life on Earth, while it has been estimated that humanity will require a quarter(!) of all that energy by 20509. So, we are running into a similar version of the problem posed by fossil fuels: we are depending on the chemical energy of a constrained resource to power the processes we rely on. Biomaterials alone cannot act as a sustainable fossil fuel replacement, and no other chemical energy sources are replenished continuously the way that biological resources are by sunshine10. What if we abandon chemical energy altogether as our energy source?
Electricity is an extremely convenient and accessible form of energy. Historically, the energy to generate electricity originated from the combustion of fossil fuels, but luckily, things have changed dramatically in the last several years. Renewable energy is making up more and more of the electricity on the grid11 due to sharply decreasing costs in solar and wind power production12, with solar energy growing especially fast, going from 0.6% to 5.5% of global electricity production within the past decade13.
Renewable energy is making up more and more of the electricity on the grid due to sharply decreasing costs in solar and wind power production, with solar energy growing especially fast, going from 0.6% to 5.5% of global electricity production within the past decade
Now, we can use electricity to power chemical processes instead
Now, thanks to the pioneering breakthroughs in electrochemistry by Twelve's founders, and the work we do here, we can use electrical power generated from renewable sources to power chemical processes.
This is a real and achievable way of transitioning from limited chemical resources, as the limit now expands to the energy we can harvest from sunshine and wind independently of arable lands and petroleum reservoirs. This solves one of the most critical issues with traditional chemical processes in that it enables powering them without depleting a constrained chemical resource. Although it is possible to power thermochemical reactions with renewable electricity through electric heating, Twelve is moving beyond thermochemistry as we seek to harness the sustainability benefits of electrochemistry. Electrochemistry is the form of chemistry where electrical energy is directly converted into chemical energy or vice versa. This opens up the door for more energy-efficient chemistry. Why so? Well, every time energy is converted into a different form, such as from electricity to heat and then from heat to chemical energy as is the case for renewable-powered thermochemistry, we lose some of it. This energy loss is unavoidable, so it is generally best to minimize the number of times we convert energy into a different form to make a process as energy-efficient as possible, especially considering the many terawatts of power needed to supply the world’s needs sustainably14.
Electrochemistry is the form of chemistry where electrical energy is directly converted into chemical energy or vice versa
With electrochemistry, we skip the intermediate step of heat energy altogether and instead convert the electrical energy into chemical energy in a single step. Shortcutting directly from electrical to chemical energy decreases the opportunities for energy to be lost in the overall process, allowing us to achieve higher energy efficiencies.
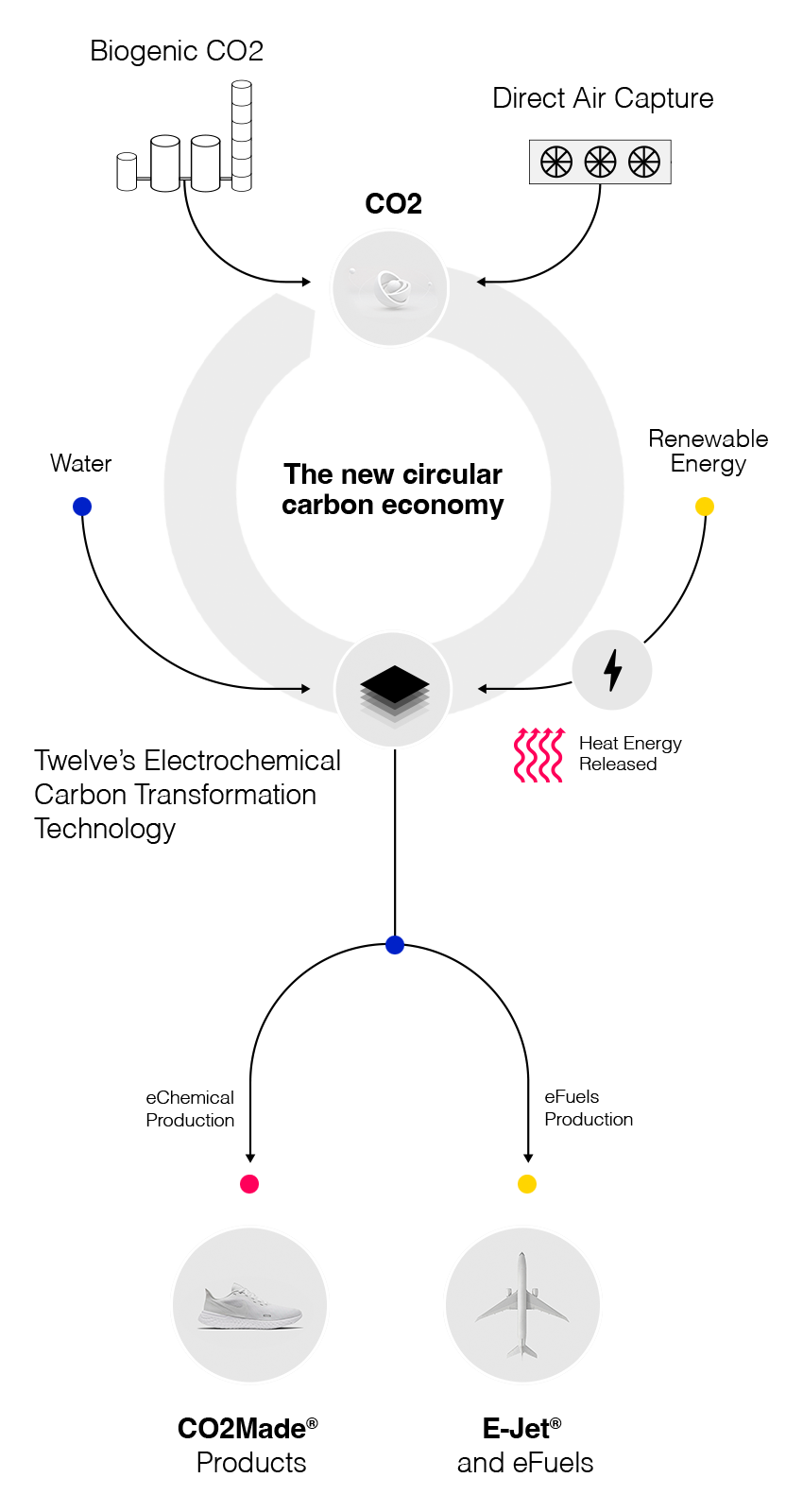
For example, hydrogen production from water, where both the thermochemical and electrochemical reaction have been heavily researched, has exhibited twice as high energy efficiency with the electrochemical version compared to the thermochemical one15. To run an electrochemical reaction, a reactor is connected to electricity. Once the electrical current is switched on, one end of the reactor becomes positively charged and the other end negatively charged. This creates an asymmetry that is not present in thermochemical reactors. Thermochemical reactors have molecules reacting with each other throughout the whole volume of the reactor, while electrochemical reactors (also called electrochemical cells) split each reaction into so-called half-reactions, with one half-reaction occurring on the positive side and the other half-reaction on the negative side (named the positive and negative electrode, respectively).
Let’s look at CO2 reduction (CO2 → CO + 1⁄2O2) as an example. In a thermochemical reactor, the reaction happens throughout the volume of the reactor (CO2 + heat → CO + 1⁄2O2), while in an electrochemical reactor, the reaction is split into the half-reactions CO2 + H2O + 2e- → CO + 2OH- on the negative electrode and 2OH- → 1⁄2O2 + H2O + 2e- on the positive electrode16. The e- in the half-reactions represents an electron, which is the negatively charged particle that makes up the bonds between atoms in molecules. Since electrical current is simply the flow of charges such as electrons and ions, the act of running electrical current through the cell causes electrons to be withdrawn from OH- on the positive electrode and flow through the wire towards the negative electrode where electrons are pushed into CO2 to turn it into CO. Inside the reactor, the OH- ions that are generated on the negative electrode flow through the cell towards the positive electrode, where these ions supply the electrons that run through the wire, allowing the CO production to continue.
All of this means that the rate of chemical reactions in the reactor is determined by how much electrical current we push through the cell, so doubling the chemical production rate can be as simple as doubling the electrical current. It also means that the chemical production can be immediately started and stopped with the flip of a light switch17. This is a fundamental difference from thermochemical reactors, which may take hours to turn on or off due to how long it takes them to heat up and cool down18. As a result, electrochemical reactors have the unique advantage that they can adjust their chemical production instantly on demand, which makes them well-equipped to handle the inherently intermittent nature of solar and wind power generation19.
As a side effect of the chemical reaction rate being linked to the electrical flow of current, the chemical production rate arises from the two-dimensional (2D) area available for chemical reactions on each electrode. This 2D nature of electrochemical reactors makes it possible to stack multiple electrochemical cells together where the positive end of one cell is electrically connected to the negative end of the next cell. Since the chemical production rate scales with the number of cells, you can stack together as many or as few cells as you want to match your needs. You might wonder, can’t thermochemical reactors also be tailored to the size you need? Not quite. The problem there is the high temperatures; thermochemical reactors lose heat faster if they are made smaller due to the higher surface-to-volume ratio, which leads to more wasted energy. Since electrochemical reactors do not rely on heat as their energy input, they can maintain the same energy efficiency, and thus also cost, regardless of scale. They can therefore be economically viable at smaller scales that are unfeasible for thermochemical systems20. This enables decentralized chemical manufacturing, opening up the door to production of e.g., jet fuel at airports21 and fertilizers in remote agricultural areas22. By building out a network of many small-scale production facilities instead of a few large ones, we no longer need to transport chemicals for long distances to where they are needed, decreasing energy consumption due to fuels for transportation and also lowering emissions23.
To summarize our story so far, employing renewable electricity as the energy input for chemical reactions resolves the issue of constrained energy supply from fossil fuels and biomaterials. This electrical energy can be converted into heat to power thermochemical reactions for sustainable chemical production, but this “energy detour” (electricity → heat → chemical) leads to additional energy waste which we may not be able to afford due to the high projected energy needs on a global scale. Electrochemistry powered by renewable electricity offers a more energy-efficient alternative, where applying electrical energy directly into chemical energy decreases the energy waste. It also provides further inherent strengths for sustainable chemical production due to its suitability for integration with intermittent renewable electricity and its potential to unlock decentralized chemical manufacturing.
Electrochemistry powered by renewable electricity offers a more energy-efficient alternative, where applying electrical energy directly into chemical energy decreases the energy waste. It also provides further inherent strengths for sustainable chemical production due to its suitability for integration with intermittent renewable electricity and its potential to unlock decentralized chemical manufacturing
Several electrochemical technologies have already successfully entered the market and proven superior to their equivalent thermochemical technologies. One example is the lithium-ion battery, which since the 1990s has proven to be the superior choice in numerous applications, including cell phones24, cars25, and energy storage for solar power plants26. Another vital but less famous example is the chlor-alkali process, which has been well-established in producing chlorine and caustic soda on the megaton scale for several decades now27.
New applications are explored as the field of electrochemistry expands, including wastewater treatment28, solar cell production29, fertilizer30, cement31, steel32, and CO2 direct air capture33. The horizon for what is possible grows day by day, and Twelve is at the forefront of this electrochemical revolution that will transform chemical manufacturing for the next century.
2. 2019. U.S. Department of Energy. November 2019.
3. published, Michael Schirber. 2009. “The Chemistry of Life: Oil’s Many Uses.”
Livescience.com. July 20, 2009. https://www.livescience.com/5574-chemistry-life-oil.html.
4. CO2 is the most stable form of carbon when oxygen is present, which is the case most of the time since air contains oxygen. If oxygen is absent, then graphite is the most stable form. Graphite exposed to oxygen at high enough temperature will convert it into CO2.
5. Marxer, Daniel, Philipp Furler, Michael Takacs, and Aldo Steinfeld. 2017. “Solar
Thermochemical Splitting of CO2 into Separate Streams of CO and O2 with High Selectivity,
Stability, Conversion, and Efficiency.” Energy & Environmental Science 10 (5): 1142–49.
6. admin. 2020. “Glassblowing FAQs | Epiphany Studios Blog | Art | Artist.” Epiphany Studios. July 31, 2020. https://epiphanyglass.com/glassblowing-faqs.
7. Solar thermal is also a possibility for generating the heat needed but requires large areas of land to concentrate enough sunlight to get to the relevant temperatures so it can only be
applied in large-scale factories in areas with sufficient direct sunlight: Wang, Z., R.R.
Roberts, G.F. Naterer, and K.S. Gabriel. 2012. “Comparison of Thermochemical,
Electrolytic, Photoelectrolytic and Photochemical Solar-To-Hydrogen Production
Technologies.” International Journal of Hydrogen Energy 37 (21): 16287–301.
8. “Earth’s Energy Flow - Energy Education.” 2018. Energyeducation.ca. 2018.
9. Kurtz, Sarah R., Ashling (Mehdi) Leilaeioun, Richard R. King, Ian Marius Peters, Michael J. Heben, Wyatt K. Metzger, and Nancy M. Haegel. 2020. “Revisiting the Terawatt Challenge.”MRS Bulletin 45 (3): 159–64. https://doi.org/10.1557/mrs.2020.73.
10. Energy.Gov. n.d. “Energy Sources.” Energy.gov. https://www.energy.gov/energy-sources.
11. US EPA. 2022. “U.S. Electricity Grid & Markets.” Www.epa.gov. January 10, 2022.
12. Armstrong, Martin. 2021. “The Price of Solar Power Has Fallen by over 80% since 2010.
Here’s Why.” World Economic Forum. November 4, 2021.
13. “Share of Electricity Production from Solar.” n.d. Our World in Data.
14. Kurtz, Sarah R., Ashling (Mehdi) Leilaeioun, Richard R. King, Ian Marius Peters, Michael J. Heben, Wyatt K. Metzger, and Nancy M. Haegel. 2020. “Revisiting the Terawatt Challenge.” MRS Bulletin 45 (3): 159–64. https://doi.org/10.1557/mrs.2020.73.
15. Vidas, Leonardo, and Rui Castro. 2021. “Recent Developments on Hydrogen Production
Technologies: State-of-The-Art Review with a Focus on Green-Electrolysis.” Applied
Sciences 11 (23): 11363. https://doi.org/10.3390/app112311363.
16. This is just one example of paired half-reactions from CO2 → CO + ½O2; others are possible too, and which pair of half-reactions that occur in the cell is a result of how the reactor is designed.
17. Buttler, Alexander, and Hartmut Spliethoff. 2018. “Current Status of Water Electrolysis for
Energy Storage, Grid Balancing and Sector Coupling via Power-To-Gas and Power-To-
Liquids: A Review.” Renewable and Sustainable Energy Reviews 82 (3): 2440–54.
18. Cornelius, R, and Hodel A E. 1985. “Reactor Cooldown Time Cut 30-50% with Liquid
Nitrogen Injection.” Chem. Process. (Chicago); (United States) 48:10 (August).
19. Buttler, Alexander, and Hartmut Spliethoff. 2018. “Current Status of Water Electrolysis for
Energy Storage, Grid Balancing and Sector Coupling via Power-To-Gas and Power-To-
Liquids: A Review.” Renewable and Sustainable Energy Reviews 82 (3): 2440–54.
20. Xia, Rong, Sean Overa, and Feng Jiao. 2022. “Emerging Electrochemical Processes to
Decarbonize the Chemical Industry.” JACS Au 2 (5): 1054–70.
21. Pollution Solutions. 2019. “Rotterdam the Hague Airport Initiates Study for the Production of Renewable Jet Fuel from Air.” Pollution Solutions Online. Pollution Solutions. 2019. https://www.pollutionsolutions-online.com/news/air-clean-up/16/edl-anlagenbau-gesellschaft-mbh/rotterdam-the-hague-airport-initiates-study-for-the-production-of-renewable-jet-fuel-from-air/49383.
22. Li, Wenyi, Ke Li, Yixing Ye, Shengbo Zhang, Yanyan Liu, Guozhong Wang, Changhao Liang, Haimin Zhang, and Huijun Zhao. 2021. “Efficient Electrocatalytic Nitrogen Reduction to Ammonia with Aqueous Silver Nanodots.” Communications Chemistry 4 (1): 1–11.
23. Martín, Antonio José, and Javier Pérez-Ramírez. 2019. “Heading to Distributed
Electrocatalytic Conversion of Small Abundant Molecules into Fuels, Chemicals, and
Fertilizers.” Joule 3 (11): 2602–21. https://doi.org/10.1016/j.joule.2019.09.007.
24. Amit Katwala. 2018. “The Explosive Race to Totally Reinvent the Smartphone Battery.”
WIRED. WIRED. July 30, 2018. https://www.wired.com/story/smartphone-battery-life-lithium-ion-future/.
25. “Evolute Group.” 2023. Evolute - Evolute. September 29, 2023. https://www.evolute.in/e-mobility-revolution-lithium-ion-batteries-powering-the-transportation-industry/.
26. “Batteries for Renewable Energy Storage.” n.d. Www.iec.ch.
27. Review of 8.11 Chlor-Alkali. 1993. In AP 42, Fifth Edition, Volume I. U.S. Environmental
Protection Agency. https://www.epa.gov/air-emissions-factors-and-quantification/ap-42-fifth-edition-volume-i-chapter-8-inorganic-1.
28. “A Sustainable Approach to Managing High- Strength Wastewater.” n.d. Accessed October 23, 2024.
https://info.aquacycl.com/hubfs/Sustainable_Approach_to_High_Strength_Wastewater_whitepaper_Aquacycl_April2021.pdf.29. Yasuda, Kouji and Nohira, Toshiyuki. "Electrochemical production of silicon" High Temperature Materials and Processes 41, no. 1 (2022): 247-278.
30. Rodrigues, Mariana, R. Jensen Lund, Annemiek ter Heijne, Tom Sleutels, Cees J. N.
Buisman, and Philipp Kuntke. 2022. “Application of Ammonium Fertilizers Recovered by an
Electrochemical System.” Resources, Conservation and Recycling 181 (June): 106225.
31. Bahnasawy, Nour, Sara Al Anany, and Nageh K. Allam. 2024. “Electrochemical Catalysis for the Production of Green Cement: Towards Decarbonizing the Cement Industry.” Catalysis Science & Technology 14 (15): 4087–4105. https://doi.org/10.1039/d4cy00009a.
32. Lopes, Daniela V., Margarida J. Quina, Jorge R. Frade, and Andrei V. Kovalevsky. 2022.
“Prospects and Challenges of the Electrochemical Reduction of Iron Oxides in Alkaline
Media for Steel Production.” Frontiers in Materials 9 (September).
33. Rahimi, Mohammad, Aliza Khurram, T. Alan Hatton, and Betar Gallant. 2022.
“Electrochemical Carbon Capture Processes for Mitigation of CO2 Emissions.” Chemical
Society Reviews 51 (20): 8676–95. https://doi.org/10.1039/d2cs00443g.